Perinatal Omega-3 Deficiency May Condition Future Sensitivity to Food Reward and Impaired Energy Expenditure
This article at a glance
Conclusive experimental support for this fundamental idea is nearly impossible to obtain in human studies, given the length of the study needed, but could be tested in animal models. A recent study in mice has taken an experimental approach to examine whether the perinatal consumption of omega-3 long-chain PUFA (omega-3 LCPUFA) can modify the behavior of progeny as they mature into adulthood, and bias it towards harmful rewards that would make them prone to develop obesity. To test this hypothesis, the study examined whether perinatal omega-3 LCPUFA deficiency would make mice more likely to respond to a sugar reward, a type of behavior that could lead to increased weight. In addition, the study also assessed several other parameters related to weight gain, such as mobility and emotional control.
The study was carried out by Auguste and colleagues at the Montreal Diabetes Research Center at the Centre de Recherche du Centre Hospitalier de l'Université de Montréal, the Department of Nutrition, Faculty of Medicine at Montreal University, and the Montreal Heart Institute, Québec, Canada. Pregnant mice were divided into two groups that were fed an omega-3 LCPUFA deficient diet or an omega-3 LCPUFA adequate diet. A lack of omega-3s in the animal feed was created by only including safflower oil and coconut oil as the sources of fat. The diet with omega-3 LCPUFA contained docosahexaenoic acid (DHA) from algae and α-linolenic acid (ALA) from flaxseed oil, replacing part of the safflower and coconut oils. The dietary composition of both mouse diets was otherwise the same and isocaloric. Access to the diets was continued up to 3 weeks after birth, so that both the mothers (dams) and the pups received the assigned diets after birth. When the pubs were weaned at 3 weeks they were all given access to a normal mouse feed. At the age of 6 weeks, the male mice were separated into two groups and given either a high fat diet rich in saturated fatty acids or a normal mouse diet. The brains of the female mice were analyzed at 6 weeks for their lipid and fatty acid composition (the researchers already knew that no difference in fatty acid composition is detectable between male and female mice). The male mice were used for the following functional experiments. Food intake and body weight were measured daily between 6 and 20 weeks of age. Fat and lean mass was determined at 20 weeks non-invasively by echo magnetic resonance imaging. These measurements were complemented at week 21 with a recording of locomotor activity (at the beginning of the night, when mice are most active). A specific test assessed refeeding following caloric restriction, in mice that were 8-9 weeks old. Daily food intake was first determined precisely, followed by providing individual mice only half of their daily food intake, for 3 days. After this restriction, the mice were given free access to food again and food intake was measured up to 8 hours.
Another test measured the rewarding effect of sucrose (i.e. common sugar) in the same male mice one week later. Mice were first trained to press a lever upon which in return they automatically received a small sucrose pellet. This type of learning following a specific reward is called operant behavior. Pressing the response lever (“the operandum”) was recorded. By modulating the reward frequency for each reinforced behavioral action, behavioral scientists can study the level of motivation for a specific reward. A specific operant procedure known as the progressive ratio schedule of reinforcement requires the mouse to make an increasing number of lever presses for each successive sucrose pellet reward. The number of responses made to obtain the last reward is termed the breakpoint and serves as an index of reward strength. Finally, two tests for innate anxiety-related behavior were carried out. In the first, the “elevated plus maze”, the mice are given the choice between exploring areas (“arms”) which are elevated from the floor and protected with high walls, or without walls. The innate response of mice is to spend most time in the enclosed arms and an aversion to venture out into the unprotected arms of the maze or even close to these, at the center of the maze. A reduction in the number of exploratory entries into the elevated open arms of the maze is a measure of the level of anxiety. In the second anxiety test, the time spent by a mouse in the center of an open area (“open field”) is measured. The natural tendency of mice is to explore new areas but to avoid open spaces. Shorter times spent at the center of the open area are taken as an indication of higher anxiety. Perinatal omega-3 LCPUFA deficiency led to changes in the fatty acid composition of brain tissue of the 6-week old mice. A statistically not significant decrease in DHA and total omega-3 LCPUFA was observed (DHA comprises >90% of the total mass of omega-3 LCPUFA in murine brain tissue). Other omega-3 LCPUFA species in brain tissue, including docosapentaenoic acid (DPA) n-3, did not significantly decrease, but EPA levels decreased 10-fold (from 0.018 % to 0.0018% of total fatty acid). There was a significant ~25% increase in the level of arachidonic acid, and both the ratio of DHA to AA, and that of total omega-3 LCPUFA to omega-6 LCPUFA, were lower, from about 2.1 in control mice, to 1.3 in mice from deficient dams. Mice from the omega-3 LCPUFA-deficient dams displayed a significant increase (~12%) in food intake following the caloric restriction challenge, compared to control mice, but only at the 8-hour timepoint. In the test for operant behavior, the two groups of mice did not show a difference in learning behavior on 8 consecutive lever-pressing training days. After having learned the goal-oriented behavior, the mice from the omega-3 LCPUFA-deprived mothers displayed a two-fold increase in breakpoint ratio, i.e. they were much more motivated to press the lever for a much greater number of times to obtain the sucrose pellet reward. The omega-3 deficient offspring grew significantly faster than control mice from week 12 onwards on the high-fat diet. Mice eating the standard feed gained weight at a slower pace, and no significant difference was seen between the two types of mice, except at week 20, when the deficient mice had attained a small but significantly higher body weight. The increased body weight was principally seen as increased fat mass. Lean mass also showed a small but significant elevation in the mice from omega-3 LCPUFA-deprived dams, both on the normal and high-fat mouse diets.
Although a relative body weight increase was observed in the omega-3 LCPUFA-deficient mice, this was not attributable to increased food intake; the cumulative food intake, measured as kcal over the 14-week period, was the same for both groups of mice on either diet type. This somewhat surprising result led to the explanation that increased body weight could have resulted primarily from the lower locomotor activity of the omega-3 LCPUFA-deficient mice compared to the control mice. In fact, on the high-fat diet, the mice moved half as much as the control mice. This “sedentary” behavior was also observed in the omega-3 LCPUFA-deficient mice on the standard feed, although it was less pronounced. The omega-3 LCPUFA-deficient mice spent about half the time at the center of the open field compared to control mice, irrespective of diet type. In this model, mice that had received the high-fat feed during their adult life displayed anxious behavior that was already twice as intense compared to the mice that had eaten a normal mouse feed. The results of the elevated plus maze showed that high-fat feeding resulted in a lower number of open arm entries and reduced time spent in the open arms. However, no clear effects of perinatal omega-3 deficiency were seen in this model. These results suggest that perinatal omega-3 LCPUFA deficiency may have long-lasting effects on specific aspects of anxiety, as well as on the predisposition to develop anxiety-like behavior when eating a diet with an elevated fat content. In summary, this study has provided experimental evidence that supports the hypothesis that perinatal omega-3 LCPUFA deficiency imparts several intricate body weight-related effects in offspring that can last into adulthood. Specifically, omega-3 deficiency around birth heightened the rewarding effects of sucrose in later life, led mice to eat more after a period of food restriction, and this may facilitate diet-induced weight gain by reducing physical motility. These effects were accompanied by changes in brain polyunsaturated fatty acid levels, and possibly by heightened anxiety. Some of these effects were more pronounced when a high-caloric diet was provided during adult life. Perinatal omega-3 deficiency in mice is a model that has long been used to study the effects of omega-3 LCPUFA deficiency. Exclusion of omega-3 LCPUFA from the diet during gestation is well known to cause behavioral deficits, at least in animal models. Long-term exposure to an omega-3 deficient diet is also known to impair the cannabinoid receptor signaling pathway in mood-controlling brain structures. The study did not address if the extent of omega-3 LCPUFA level reduction was of sufficient magnitude to affect vision and motor function, which are other known developmental defects resulting from omega-3 deficiency. It would be interesting to determine if these defects contributed to the reported outcomes (e.g. lever pressing, mobility and anxiety). Changes in brain fatty acid levels were measured in total brain tissue, but more subtle effects in specific brain regions were thus undetected or may be even larger than the average values determined in this study. Although EPA is a minor fatty acid in brain tissue, the marked effect of perinatal omega-3 deficiency on its tissue level merits further investigation. No report was made of the tissue level of C22:5 n-6 (docosapentaenoic acid n-6), a fatty acid which could be expected to increase in tissue level to compensate for a decrease in DHA under conditions of omega-3 LCPUFA deficiency. A major limitation of this study is that no information was provided on the fatty acid composition of the control diet and the “high fat” diet. For example, the safflower oil could be a commodity oil or a high-oleic oil. Knowing the precise composition of fatty acids is critical for these type of studies, since individual fatty acids can exert specific effects on feeding behavior. For example, linoleic acid intake perinatally is known to markedly affect later life emotional behavior of mice. Human clinical evidence supporting the hypothesis that consuming more omega-3 LCPUFA during gestation enhance human responses to harmful rewards has not yet been explicitly reported. However, lower seafood intake during pregnancy is associated with a higher risk of neurodevelopmental abnormalities in children, such as suboptimum outcomes for socialising behavior, and lower fine motor, communication, and social development scores. Prospective population studies have provided indications that omega-3 LCPUFA intake associated with fish consumption or supplementation during pregnancy benefits the neurocognitive development and problem-solving capacity of young children. An important role of fatty acids in fetal programming has been recognized, and fits into the theory of the “thrifty gene”, which states that in utero programming provides a means to adapt fetal tissue development to the environmental conditions a pregnant mother experiences and which the developing child is expected to experience in its future adult life. A low perinatal intake of omega-3 LCPUFA may thus constitute one of the important environmentally-determined dietary factors that is integrated into the cognitive development that determines the future dietary habits of a person. Effects of omega-3 status during gestation on different aspects of human physiology later in life have been investigated, although such studies are limited mostly to infants, children and teenagers/young adults. For example, the supplemental intake of fish oil during pregnancy may reduce asthma incidence in 3-5-year old children, an effect that is related to blood omega-3 LCPUFA levels, and may even provide beneficial effects until age 16. Studies that have addressed the role of maternal omega-3 status and diet during pregnancy on the risk for obesity development have not provided unambiguous results, and further research is necessary. One recent study has shown that increased body mass index in 2- and 4-year-old children was significantly associated with lower maternal red blood cell DHA levels at 36 weeks gestation, suggesting that it is important to link the outcomes to omega-3 status. Although omega-3 LCPUFA supplementation during pregnancy has recently been shown to increase LCPUFA-derived Specialized Proresolving Mediator levels at birth, their significance for long term modification of health and disease risk remains to be established.
Based on the relatively new insight that developmental epigenetic programming may influence life-long health and disease risk, researchers have addressed the influence of maternal omega-3 LCPUFA intake and status on the offspring’s global DNA and sequence-specific epigenetic modifications. It is now known that dietary omega-3 LCPUFA intake during pregnancy exerts an intrauterine epigenetic effect, which can be measured as changes in the DNA methylation state in, for example, immune cells in neonates, and which can persist until at least age 5. Maternal omega-3 LCPUFA levels also rank among the most relevant maternal metabolites driving epigenetic programming, as concluded from their influence on infant cord blood DNA methylation. Dietary modification during the perinatal period is now viewed to be a useful starting point in the primary prevention of cognitive health problems of offspring, but whether obesity development in children and later life can be modulated through perinatal dietary behavior remains to be formally demonstrated. In summary, the present study has provided experimental support that perinatal levels of omega-3 LCPUFA may be involved in determining the reward behavior of easily palatable foods in the offspring’s later life. If extrapolated to the human situation these results could mean that inadequate perinatal omega-3 LCPUFA intake may set up infants to develop into individuals that are sensitive to gaining additional weight later in life because their brain reward system is primed to seeking palatable food. That would constitute a tragic consequence in the context of the nearly unrestricted abundance of high energy-containing foods easily available to most people today. New research is needed to confirm the findings of the current study, particularly with respect to the precise fatty acid composition of the animal diets, and to determine if this specific long-lasting aspect of diet quality is indeed of relevance to the human situation. Auguste S, Sharma S, Fisette A, Fernandes MF, Daneault C, Des Rosiers C, Fulton S. Perinatal deficiency in dietary omega-3 fatty acids potentiates sucrose reward and diet-induced obesity in mice. Int. J. Dev. Neurosci. 2017;Sept. 15. [PubMed] Worth Noting Avon Longitudinal Study of Parents and Children ASPAC: http://www.bristol.ac.uk/alspac/ Bisgaard H, Stokholm J, Chawes BL, Vissing NH, Bjarnadottir E, Schoos AM, Wolsk HM, Pedersen TM, Vinding RK, Thorsteinsdottir S, Folsgaard NV, Fink NR, Thorsen J, Pedersen AG, Waage J, Rasmussen MA, Stark KD, Olsen SF, Bonnelykke K. Fish oil-derived fatty acids in pregnancy and wheeze and asthma in offspring. N. Engl. J. Med. 2016;375(26):2530-2539. [PubMed] Blumfield ML. Can long-chain PUFA supplementation during pregnancy influence later obesity risk? Am. J. Clin. Nutr. 2016;103(6):1387-1388. [PubMed] Brenna JT. Animal studies of the functional consequences of suboptimal polyunsaturated fatty acid status during pregnancy, lactation and early post-natal life. Matern. Child Nutr. 2011;7(Suppl. 2):59-79. [PubMed] Echo magnetic resonance imaging - https://cfmi.georgetown.edu/downloads/training/2/MRI-basics.pdf Emmett PM, Jones LR, Golding J. Pregnancy diet and associated outcomes in the Avon Longitudinal Study of Parents and Children. Nutr. Rev. 2015;73(Suppl. 3):154-174. [PubMed] Foster BA, Escaname E, Powell TL, Larsen B, Siddiqui SK, Menchaca J, Aquino C, Ramamurthy R, Hale DE. Randomized controlled trial of DHA supplementation during pregnancy: Child adiposity outcomes. Nutrients 2017;9(566):1-10. [PubMed] Hibbeln JR, Davis JM, Steer C, Emmett P, Rogers I, Williams C, Golding J. Maternal seafood consumption in pregnancy and neurodevelopmental outcomes in childhood (ALSPAC study): an observational cohort study. Lancet 2007;369(9561):578-585. [PubMed] Kabaran S, Besler HT. Do fatty acids affect fetal programming? J. Health Popul. Nutr. 2015;33(14). [PubMed] Larrieu T, Madore C, Joffre C, Layé S. Nutritional n-3 polyunsaturated fatty acids deficiency alters cannabinoid receptor signaling pathway in the brain and associated anxiety-like behavior in mice. J. Physiol. Biochem. 2012;68(4):671-681. [PubMed] Lee HS, Barraza-Villarreal A, Biessy C, Duarte-Salles T, Sly PD, Ramakrishnan U, Rivera J, Herceg Z, Romieu I. Dietary supplementation with polyunsaturated fatty acid during pregnancy modulates DNA methylation at IGF2/H19 imprinted genes and growth of infants. Physiol. Genomics 2014;98(2):480-487. [PubMed] Lillycrop KA, Burdge GC. Epigenetic mechanisms linking early nutrition to long term health. Best Pract. Res. Clin. Endocrinol. Metab. 2012;26(5):667-676. [PubMed] Montreal Diabetes Research Center: http://www.montreal-diabetes-research-center.org/en/index.asp Moriguchi T, Greiner RS, Salem N, Jr. Behavioral deficits associated with dietary induction of decreased brain docosahexaenoic acid concentration. J. Neurochem. 2000;75(6):2563-2573. [PubMed] Olsen SF, Østerdal ML, Salvig JD, Mortensen LM, Rytter D, Secher NJ, Henriksen TB. Fish oil intake compared with olive oil intake in late pregnancy and asthma in the offspring: 16 y of registry-based follow-up from a randomized controlled trial. Am. J. Clin. Nutr. 2008;88(1):167-175. [PubMed] O'Neil A, Itsiopoulos C, Skouteris H, Opie RS, McPhie S, Hill B, Jacka FN. Preventing mental health problems in offspring by targeting dietary intake of pregnant women. BMC Med. 2014;12(208). [PubMed] Progressive-ratio responding for palatable high-fat and high-sugar food in mice : Video - https://www.jove.com/video/3754/progressive-ratio-responding-for-palatable-high-fat-high-sugar-food Sakayori N, Tokuda H, Yoshizaki K, Kawashima H, Innis SM, Shibata H, Osumi N. Maternal nutritional imbalance between linoleic acid and alpha-linolenic acid increases offspring's anxious behavior with a sex-dependent manner in mice. Tohoku J. Exp. Med. 2016;240(1):31-37. [PubMed] See VHL, Mas E, Prescott SL, Beilin LJ, Burrows S, Barden AE, Huang RC, Mori TA. Effects of prenatal n-3 fatty acid supplementation on offspring resolvins at birth and 12 years of age: a double-blind, randomised controlled clinical trial. Br. J. Nutr. 2017;118(11):971-980. [PubMed] Sharma S, Hryhorczuk C, Fulton S. Progressive-ratio responding for palatable high-fat and high-sugar food in mice. J. Vis. Exp. 2012;63:e3754. [PubMed] Staddon JE, Cerutti DT. Operant conditioning. Annu. Rev. Psychol. 2003;54:115-144. [PubMed] van Dijk SJ, Zhou J, Peters TJ, Buckley M, Sutcliffe B, Oytam Y, Gibson RA, McPhee A, Yelland LN, Makrides M, Molloy PL, Muhlhausler BS. Effect of prenatal DHA supplementation on the infant epigenome: results from a randomized controlled trial. Clin. Epigenetics 2016;8(114). [PubMed] Vollet K, Ghassabian A, Sundaram R, Chahal N, Yeung EH. Prenatal fish oil supplementation and early childhood development in the Upstate KIDS Study. J. Dev. Orig. Health Dis. 2017;8(4):465-473. [PubMed] Ward ZJ, Long MW, Resch SC, Giles CM, Cradock AL, Gortmaker SL. Simulation of growth trajectories of childhood obesity into adulthood. N. Engl. J. Med. 2017;377(22):2145-2153. [PubMed]
- Is it possible that the quality of food during gestation and around birth sets the stage for the eating behaviour of the offspring when they become adults?
- This study reports that in mice a perinatal deficiency in dietary omega-3 fatty acids has long-lasting effects into adulthood, enhancing the brain circuits for reward, enhancing the anxiety state and reducing spontaneous activity.
- The results provide experimental support for the hypothesis that dietary omega-3 LCPUFA deficiency during pregnancy increases the motivation to eat easily palatable foods that may promote negative emotions, which can further promote overeating and obesity.
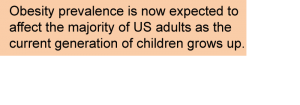
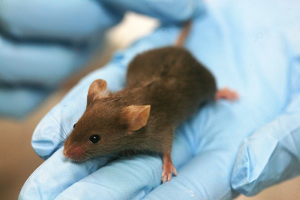
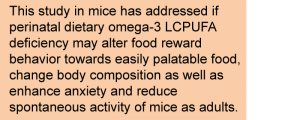
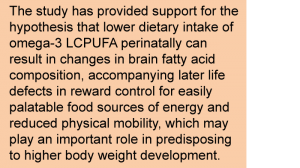
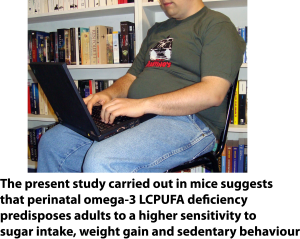